Battery-electric vehicles (BEVs) are, therefore, considered by many to have a somewhat limited role in the decarbonisation of large commercial vehicles. It is often suggested that hydrogen is the only viable route to decarbonise this transport sector.
Others argue that the significantly lower energy efficiency of fuel-cell electric vehicles (FCEVs) means they make little sense when we’re already struggling to establish enough renewable generation to replace fossil fuels. Electric road systems are a potentially more efficient and economical option, although one for which the economics are yet to be proven. This article explores these different power options.
Feasibility of BEVs
For logistical reasons, heavy vehicles are often expected to make long journeys as quickly as possible. EU rules allow a single driver to drive for 4.5 hours, take a 45-minute break, and then continue for another 4.5 hours. At the normal UK speed limit for these vehicles of 60mph, this equates to a range of 270 miles for each 4.5-hour leg. The Tesla Semi is being advertised with a range of 500 miles, which would make such journeys feasible with fast charging during the 45-minute break.
So it would seem that converting heavy vehicles to BEVs might be a feasible option after all. Although perhaps not for longer continuous journeys, where two drivers could extend the driving range considerably.
The biggest challenges for the widespread adoption of BEVs are the cost and resource dependence of batteries. Cost may actually be less of an issue for commercial vehicles than for private cars. Owing to their much higher utilisation, commercial vehicles are able to recover the increased capital cost though lower running costs more rapidly.
The use of critical metals in battery production may be a more significant issue. Meeting targets for BEV production using current battery chemistries would require the global supply of a number of critical metals to increase by multiples of current production levels.
This would mean bringing new mines online rapidly, in some cases in locations that are beyond current technical and commercial capabilities, such as deep-sea mining of manganese nodules. For example, cobalt is normally extracted as a by-product of mining other metals, such as copper, nickel or silver, and economic extraction is therefore tied to demand for these other metals. Land-based cobalt reserves are not easily extracted. Even with an immediate and massive effort to scale up mining operation to the required level, this would take at least 10 years.
There are further commercial pressures that make rapidly expanding mining operations outside centrally controlled economies such as China difficult. Subsidies provided to mining companies in China make it difficult for operators in other territories to expand in a commercially viable way. Even when other countries do offer subsidies, the timeline for opening a mine is three or four times the life of a democratically chosen government, which creates significant commercial uncertainty.
Efficiency of FCEVs
Starting with a consideration of only the hydrogen consumption within the vehicle itself, current FCEVs use approximately twice as much energy as BEVs, owing to the 40% to 50% energy efficiency of proton exchange membrane fuel cells. More efficient alkaline or solid-oxide fuel cells cannot be used in vehicles owing to lengthy start-up times, a lack of responsiveness and, in the case of solid oxide, high operating temperatures.
Considering the energy efficiency of the vehicle itself is less than half the picture. Assuming that green hydrogen from renewable sources is used, the renewably generated electricity must first be used to produce hydrogen by the electrolysis of water. The most efficient electrolysers can produce hydrogen from water with an energy efficiency of almost 80%, but commercial operations more typically achieve around 50%. Further energy losses occur when storing and transporting hydrogen.
The standard pressure for vehicle fuel tanks is becoming established as 70MPa. The energy lost in compressing the hydrogen to this pressure equates to a typical energy efficiency of around 91%, in terms of the chemical energy transferred to the vehicle. Further losses may be encountered when compressing hydrogen for intermediate storage, vehicular transport or when pumped along pipelines. These energy losses are significantly greater than for an electrical transmission and distribution system.
Generation capacity needed
When all the energy losses are taken into account, FCEVs require significantly more renewable energy generation than BEVs. Considering only the differences in efficiency, estimates can vary between two and four times the input energy. Establishing exactly how much more generation capacity would be required is further complicated since it will depend both on how efficiencies improve as technology develops, and on the extent to which hydrogen is generated from surplus electricity.
Wind and solar sometimes produce more energy than is demanded, and storing this energy for later use with batteries is expensive, meaning there is often a surplus. When hydrogen makes up only a small part of the energy mix, it can be produced using this surplus electricity, meaning that no additional generation capacity is required.
However, a number of trends are likely to mean that there will be insufficient surplus electricity to produce hydrogen for road transport. Firstly, demand side response will be enabled through smart devices which can use more power when supply is high and less when it is low, such as home appliances, industrial processes and battery vehicles. Secondly, more grid energy storage will be added to the energy system as renewables increase their share of generation capacity. Finally, hydrogen will be required for other uses, where there are fewer viable alternatives.
Hydrogen is currently used predominantly as a chemical precursor to produce commodities such as ammonia fertiliser and steel. According to the International Energy Agency, this use already accounts for 6% of global natural gas and 2% of global coal use. This ‘grey’ hydrogen will first need to be replaced by green hydrogen, immediately using up much of the remaining surplus electricity.
Other applications that have little alternative but to use hydrogen include high-temperature heat in industrial processes, long-term grid energy storage, and aviation fuel – whether used directly in hydrogen aircraft or as a precursor to synthetic hydrocarbon fuel production. Once these essential uses are taken into account, there is unlikely to be surplus electricity for hydrogen in applications with other options, such as road transport and domestic heating. One study found that, in an economically optimal scenario, just 14% of electricity should be converted to hydrogen.
Electric road systems
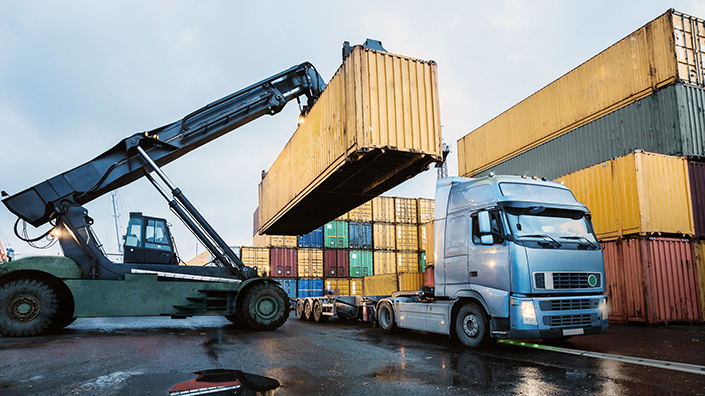
Electric road systems (ERS) provide electrical power directly to vehicles while they are driving along a road. This means vehicles can have effectively unlimited range combined with the efficiency of a fully electric drive system.
Proponents of ERS normally assume that not all roads would be fully electrified, with two scenarios generally suggested. The first scenario would involve hybrid vehicles that are directly powered by a continuous ERS on the most heavily used transport corridors, and operate using an internal combustion engine when away from the ERS. This approach is well suited to early adoption for heavy commercial vehicles, with the infrastructure on busy routes being paid for by the fuel savings achieved by large haulage companies.
The second scenario would require trunk roads to be 50% electrified in intermittent sections, with BEVs operating with greatly reduced battery capacities. This approach would be well suited to widespread use by both heavy commercial and light private vehicles. Economic studies have suggested that the cost savings from reduced battery capacities could more than offset the infrastructure cost of extensive roadway electrification. This would also greatly reduce the critical metal supply constraints that may otherwise limit the update of BEVs.
An interesting synthesis of these approaches might involve a mix of BEVs and plug-in hybrid electric vehicles. This would enable the very high efficiency, low vehicle cost and reduced critical metal dependence offered by an ERS infrastructure. At the same time, for vehicles that must access remote locations far from the ERS, internal combustion engines could eliminate range anxiety.
In such a scenario, zero emissions could still be achieved, since the high cost and energy losses of renewably produced synthetic hydrocarbon fuels could be justified for the very limited use where it would be required.
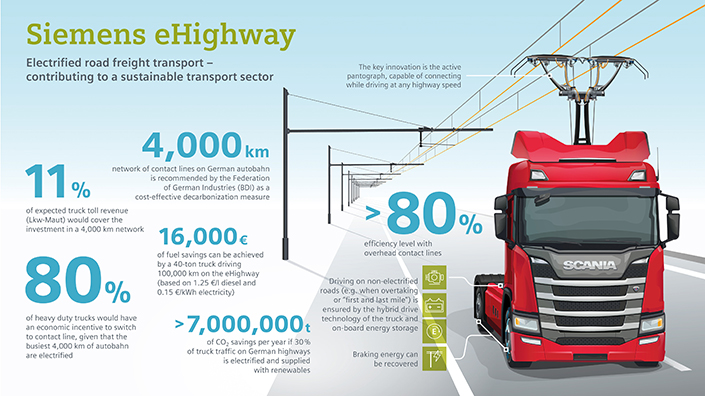
Electrical power can be provided by three different types of ERS:
Catenary systems suspend two supply lines at approximately 5m over the road. The height of the lines means that relatively long stretches of over 1km can be safely electrified. Vehicles must be equipped with a pantograph that maintains an electrical connection while allowing for lateral and vertical movements of the vehicle.
This is a mature technology which is closely related to systems used on trains, trolley buses and trams. Trials are well under way, with hybrid heavy trucks operating in fully electric mode on test sections of public highways. A 2km section has been in operation in Sweden since 2016 and a 5km section in Germany is operating on a road that sees 400,000 vehicles a day.
There are some disadvantages to catenary systems. Firstly they are only suitable for heavy commercial vehicles. Additionally, pylons on the roadside increase risk in road traffic accidents; fallen cables may endanger pedestrians; some road maintenance machinery will not fit under the wires; and there is significant visual impact.
Road-bound conductive systems place conductive tracks within the road surface or along the side of the road. This requires a much smaller pick-up that can be mounted on both heavy commercial and light private vehicles. Since these tracks are within reach of people, they are divided into short segments that are only energised when a vehicle is passing over them. An additional layer of safety may be provided by placing an earth rail at the road surface with the live rail within a deep and narrow slot. Trials of conductive track systems have been carried out primarily in Sweden.
Road-bound inductive systems place an energised coil within the road surface which induces a current in a coil on the underside of a vehicle. Dynamic inductive charging of city buses has been proven by the Dongwon Online Electric Vehicle (pictured opposite), which has operated in South Korea since 2010. Trials of dynamic inductive charging on highways have shown varied efficiencies between 66% and 87% owing to sensitivity to alignment between the coils in the road and the coils in the vehicle.
Road-bound systems require some means for vehicles to identify themselves automatically to draw power from the charging system. Identification can also allow micro-payments for electricity as it is consumed, with the standard ISO 15118 providing the required information transfer protocols.
An ERS could reduce the need for large batteries in BEVs while also greatly reducing the need for inefficient hydrogen consumption in FCEVs. This could enable more rapid decarbonisation, by lessening the effect of critical metal shortages and reducing the requirement for renewable generation capacity. At the same time, it has the potential to have the lowest societal cost of the available decarbonisation pathways for road transport.
Want the best engineering stories delivered straight to your inbox? The Professional Engineering newsletter gives you vital updates on the most cutting-edge engineering and exciting new job opportunities. To sign up, click here.
Content published by Professional Engineering does not necessarily represent the views of the Institution of Mechanical Engineers.